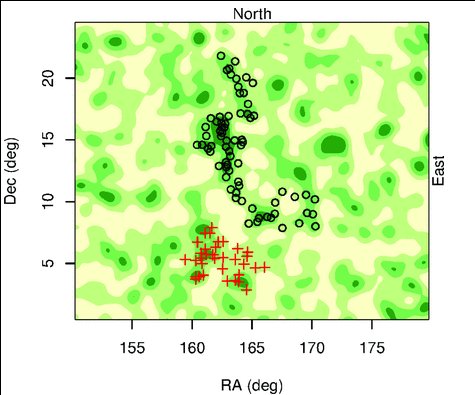
One of the more absurd assumptions that is routinely made in astronomy is called the cosmological principle. One way to phrase the principle is:
Viewed on a large enough scale, the properties of the universe are the same no matter where you are.
However, observations have never supported this assumption. Instead, the observable universe seems incredibly “lumpy,” with huge structures separated by vast areas devoid of structures. Nevertheless, cosmologists have doggedly taken the cosmological principle as their starting assumption when it comes to developing models of the universe, despite the fact that observations don’t support it.
Indeed, the cosmological principle is a necessary starting point for the Big Bang, which most, but certainly not all, astronomers think is a good description of the origin and development of the universe. As Paul Fleisher says in his book, The Big Bang:1
The cosmological principle is the central idea of the Big Bang theory. This rule says the universe is homogeneous and isotropic at very large scales.
Even if we go away from the Big Bang model, the vast majority of models that attempt to describe the universe start with the assumption that the cosmological principle is valid. There are some models that do not start with that assumption, but they are few and far between.2
I have always been skeptical of the cosmological principle, simply because it isn’t supported by observation. The universe doesn’t look homogeneous at all. Instead, it looks really “lumpy.” Nevertheless, when I read the scientific literature, the cosmological principle seems to be considered a fact in almost all of the astronomy-related papers.
It looks like that might be starting to change.
Continue reading “Astronomers are Finally Starting to Question an Absurd Assumption”